Parts 1 and 2 of this series dealt with the discovery of hybrid antibodies: fusion receptor-antibody molecules that gain reactivity against a pathogen by integrating a component of a receptor targeted by that selfsame pathogen.1,2 Although hybrid antibodies are unlikely to play a major role in most immune responses, they still represent an intriguing therapeutic opportunity for bioengineering. With the global antibody therapeutics market already worth over $250 billion USD (and projected to reach over $400 billion USD in the next five years),3 any breakthroughs that pave the way for a new first-in-class therapy have the potential to be very lucrative. To succeed, however, hybrids need to hold their own.
Hybrid versus conventional
Antibodies are a cornerstone of the modern immune system, and exist in all jawed vertebrates (i.e., all mammals and some fish).4 Having been around for much of evolutionary history, they have evolved into lots of different configurations (Figure 1).5–7 Even the few agnathans, or jawless fish, that lack true antibodies have been shown to produce antibody-like molecules called variable lymphocyte receptors B (VLR-Bs) that ‘grip’ their targets like a hand.8 Despite differences in appearance, antibodies must only do two things in order to work: 1) bind their targets (called antigens) tightly, and 2) link that binding to some functional activity.9
Conventional antibodies generally bind very tightly to their targets. This is because antibodies whose production is stimulated by the presence of antigens are refined bit-by-bit through repeated rounds of mutation in a process called somatic hypermutation. The complementarity determining region (CDR) loops that are responsible for direct contact with the antigen are relatively flexible, which means that the tips of the antibody are effectively ‘molded’ to the antigen over time.9 In principle, we would expect hybrid antibodies to bind their targets more weakly compared to their conventional counterparts for two main reasons. First, normal receptors simply bind much more weakly to their targets, or ligands, compared to antibodies.10 Second, while the flexible CDR loops are free to adopt almost any combination of mutations,9 the inserted domains of hybrid antibodies need to retain their structural integrity and so cannot change as much.1,2
Unfortunately, binding information is only available for the LAIR1 hybrids, and so caution must be exercised in generalizing from a very small sample size. Caveat aside, it turns out that the inserted LAIR1 gene is subjected to lots of mutations—much like the CDR regions would be in a normal antibody—that have the net effect of increasing the affinity for its ligand by over 150-fold.11 Researchers also found that these mutations abolished the intrinsic collagen-binding ability of the inserted domain.1 Remarkably, this means that LAIR1 hybrids walk a very fine line between balancing mutations that optimize binding (i.e., more tightly to pathogen, less tightly to collagen) while avoiding mutations that destabilize the structure of the domain which would make it come apart entirely.
There is one big problem with borrowing binding specificity from other receptors that we have yet to discuss: what prevents hybrid antibodies from binding to the normal ligand of their receptors present on healthy cells of the body (i.e., off-target effects)? This is a particularly difficult problem which the two classes of hybrids seem to have solved in different ways. As mentioned above, LAIR1 hybrids effectively repurpose a collagen-binding domain into a pathogen-binding domain through repeated mutation. The LILRB1 hybrids take a completely different approach: the inserted receptor domains originate from structural parts of the receptor, not the ligand-binding parts.2
With only one example of each hybrid that each binds to different endogenous ligands, it is difficult to say why these two approaches might exist. Since the driving force for selection of a hybrid antibody seems to be the presence of a pathogen-derived ligand, it may be down to circumstance. If the receptor’s binding site is shared by the pathogen and endogenous ligand (e.g., LAIR1), repeated mutations are necessary to minimize off-target binding. If the binding sites aren’t shared (e.g., LILRB1), self-recognition is never present in the first place.
At the end of the day, although hybrids are unlikely to be much better at binding to their targets than conventional antibodies, the fact that they are bivalent (i.e., have two binding domains) is still an important improvement over the original monovalent receptor.12 In addition, the evidence suggests that these antibodies are sufficiently active in laboratory assays to have a measurable effect, which means that the examples we have discovered so far might bind just tightly enough to work.
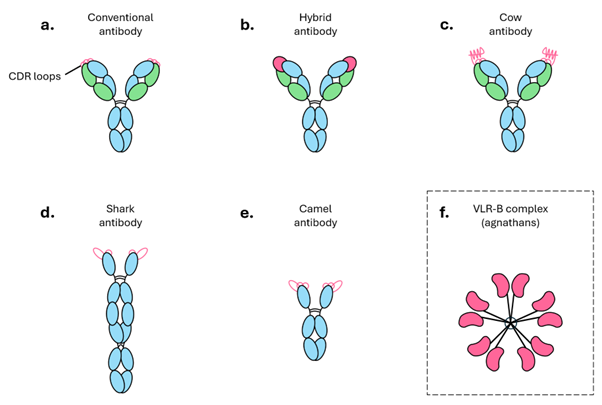
Figure 1. Antibodies come in many shapes and sizes. Schematic depiction of a) a conventional antibody consisting of two heavy chains (blue) and two light chains (green); b) a hybrid LAIR1 antibody with the receptor insert (pink) perched over the CDR loops; c) a cow antibody in which the extremely long CDR loops fold into a standalone “knob” domain; d) a shark antibody consisting of two long heavy chains and with only 2 CDR loops ; e) a camel antibody consisting of two heavy chains; f) a multimeric complex of VLR-B proteins which mediate humoral immunity in agnathans (jawless vertebrates).
Therapeutic relevance
Generating target-specific proteins de novo is very difficult,13 and so most of our efforts rely on exploiting an efficient system that already exists: screening monoclonal antibodies from immunized animals.14 Hybrid antibodies provide an opportunity for combining the methodological approach of de novo protein design with the physiological stability and bioavailability of antibodies. In theory, the only requirement for designing an artificial hybrid antibody is that the desired pathogen target binds to a well-defined (i.e., capable of folding alone) domain on an endogenous receptor. In vitro and in silico methods would be helpful in tailoring the binding profile of the antibody, which would be considerably faster than the traditional process of immortalizing antibody-producing B cells (i.e., hybridoma technology).14
Multispecific hybrid antibodies also warrant consideration. Bispecific antibodies, where each arm binds to a different target, have garnered lots of therapeutic interest recently but these artificial proteins can face issues when it comes to production, stability, and even immunogenicity—all areas where hybrids are less likely to encounter problems.15,16
Lastly, there has also been speculation about moving beyond the realm of traditional receptor-ligand interactions, for example, by building hybrids that consist of cytokine fragments to allowed cytokine signaling to be delivered in a targeted manner.17
Conclusion
Hybrid antibodies have arrived late to the stage, and so there is still much we must learn about them. This blog series has only touched on hybrids whose inserted DNA originates from conventional genes, but researchers have recently also mapped some inserts to unexpected parts of the genome: mitochondrial DNA and telomeric DNA.18 The former mostly encodes energy-generating components, enabling mitochondria to act as cellular ‘powerhouses’, while the latter has no known coding roles and acts simply as a nucleic acid buffer to delay the fraying of our chromosomes.19,20
Additionally, due to technical limitations, the current estimate of the frequency of antibody insertions (around 1 in 10,000 B cells) represents a lower limit and it is possible that they occur much more frequently.18 Finally, even if it turns out that naturally occurring hybrids are a niche mechanism to deal with very specific pathogens, their potential for biotechnological engineering should not be underestimated.
Scientific Group has extensive experience of working in immunology, including dermatology, rheumatology, gastroenterology, and oncology. If you’d like to find out more, or are looking for expert agency support in this therapy area, please contact alejandro.potes@wearescientific.com
References
- Tan J et al. A LAIR1 insertion generates broadly reactive antibodies against malaria variant antigens. Nature. 2016;529(7584):105–9.
- Chen Y et al. Structural basis of malaria RIFIN binding by LILRB1-containing antibodies. Nature. 2021;592(7855):639.
- Globe Newswire. Global Therapeutic Antibody Market Research, 2019-2023 and 2024-2029. February 27, 2024. Accessed July 4, 2024. https://www.globenewswire.com/news-release/2024/02/27/2836187/0/en/Global-Therapeutic-Antibody-Market-Research-2019-2023-and-2024-2029.html
- Hsu E. V(D)J recombination: of mice and sharks. Adv Exp Med Biol. 2009;650:166–79.
- Mills C, Campbell K. A new chapter for anti-idiotypes in low molecular weight compound immunoassays. Trends Biotechnol. 2022;40(9):1102–20.
- Wang F et al. Reshaping antibody diversity. Cell. 2013;153(6).
- Kim J et al. Globular-shaped variable lymphocyte receptors B antibody multimerized by a hydrophobic clustering in hagfish. Sci Rep. 2018;8(1):1–10.
- Boehm T et al. VLR-based adaptive immunity. Annu Rev Immunol. 2012;30:203.
- Murphy K, Weaver C. Janeway’s Immunobiology. 9th ed. Garland Science; 2017.
- Davis SJ et al. The nature of molecular recognition by T cells. Nat Immunol. 2003;4(3):217–24.
- Xu K et al. Structural basis of LAIR1 targeting by polymorphic Plasmodium RIFINs. Nat Commun. 2021;12(1).
- Oostindie SC et al. Avidity in antibody effector functions and biotherapeutic drug design. Nat Rev Drug Discov. 2022;21(10):715–35.
- Cao L et al. Design of protein-binding proteins from the target structure alone. Nature. 2022;605(7910):551–60.
- Mitra S, Tomar PC. Hybridoma technology; advancements, clinical significance, and future aspects. J Genet Engineering Biotechnol. 2021;19(1):159.
- Klein C et al. The present and future of bispecific antibodies for cancer therapy. Nat Rev Drug Discov. 2024;23(4):301–19.
- Labrijn AF et al. Bispecific antibodies: a mechanistic review of the pipeline. Nat Rev Drug Discov. 2019;18(8):585–608.
- Chen Y et al. A new mechanism of antibody diversity: formation of the natural antibodies containing LAIR1 and LILRB1 extracellular domains. Antib Ther. 2024;7(2):157–63.
- Lebedin M et al. Different classes of genomic inserts contribute to human antibody diversity. Proc Natl Acad Sci USA. 2022;119(36).
- Falkenberg M et al. Replication and transcription of human mitochondrial DNA. Annu Rev Biochem. 2024;93:47–77.
- Rossiello F et al. Telomere dysfunction in ageing and age-related diseases. Nat Cell Biol. 2022;24(2):135–47.